Pontine μ-opioid receptors mediate bradypnea caused by intravenous remifentanil infusions at clinically relevant concentrations in dogs
Abstract
Life-threatening side effects such as profound bradypnea or apnea and variable upper airway obstruction limit the use of opioids for analgesia. It is yet unclear which sites containing μ-opioid receptors (μORs) within the intact in vivo mammalian respiratory control network are responsible. The purpose of this study was 1) to define the pontine region in which μOR agonists produce bradypnea and 2) to determine whether antagonism of those μORs reverses bradypnea produced by intravenous remifentanil (remi; 0.1–1.0 μg·kg−1·min−1). The effects of microinjections of agonist [d-Ala2,N-Me-Phe4,Gly-ol5]-enkephalin (DAMGO; 100 μM) and antagonist naloxone (NAL; 100 μM) into the dorsal rostral pons on the phrenic neurogram were studied in a decerebrate, vagotomized, ventilated, paralyzed canine preparation during hyperoxia. A 1-mm grid pattern of microinjections was used. The DAMGO-sensitive region extended from 5 to 7 mm lateral of midline and from 0 to 2 mm caudal of the inferior colliculus at a depth of 3–4 mm. During remi-induced bradypnea (∼72% reduction in fictive breathing rate) NAL microinjections (∼500 nl each) within the region defined by the DAMGO protocol were able to reverse bradypnea by 47% (SD 48.0%) per microinjection, with 13 of 84 microinjections producing complete reversal. Histological examination of fluorescent microsphere injections shows that the sensitive region corresponds to the parabrachial/Kölliker-Fuse complex.
morphine and synthetic μ-opioid receptor (μOR) analogs are highly effective analgesics in humans and mammals. They can be used to treat both acute severe perioperative pain (e.g., fentanyl, sufentanil, remifentanil) and severe chronic pain states (e.g., morphine and fentanyl). The most serious, potentially life-threatening, side effect of opioids is the depression of breathing, which can occur at clinically relevant analgesic plasma concentrations. This is particularly common during recovery from surgical anesthesia, when supplemental doses of μ-opioid agonists are required for pain control and the patients' level of consciousness can frequently and rapidly alternate between consciousness and sleep/deep sedation. Profound respiratory depression is clinically manifested by marked slowing of the respiratory rate leading to bradypnea or even apnea often accompanied by upper airway obstruction. During experimentally controlled isocapnic conditions a decrease in both respiratory rate and tidal volume can be observed. These respiratory depressant effects are thought to originate within the pontomedullary respiratory rhythm and pattern-generating region and possibly in other brain stem regions including the nucleus of the solitary tract (NTS), the ventral respiratory column (VRC), and the lateral pons (Haji et al. 2003; Janczewski et al. 2002; Mansour et al. 1995; Martin-Schild et al. 1999; Sales et al. 1985). Opioid receptors have been identified on neurons throughout these nuclei, including those in the pre-Bötzinger complex (preBC), the putative locus for rhythm generation. With localized application of μOR agonists, the discharge activity of many brain stem respiratory neurons can be reduced or entirely abolished (Bianchi et al. 1995; Haji et al. 2003; Lalley 2008; Stucke et al. 2008). Since several studies have used local microinjections of μOR agonists at concentrations much greater than those obtained via systemic administration in a clinical dose range, it is possible to incorrectly assign the role of μORs in a specific location to producing an observed clinical effect. A more reliable method to evaluate the role of μORs at a specific location within the control network would be to test the ability of a locally applied antagonist to reverse the effect of the systemically applied agonist.
In vitro studies using bath application of opioids in reduced brain stem preparations (Gray et al. 1999) and studies using immunohistochemical techniques (Lonergan et al. 2003; Manzke et al. 2003) provide strong evidence for a direct opioid effect on structures within the preBC. This has led investigators to suggest that μORs in the preBC may be major contributors to the bradypnea produced by systemically administered opioids (Lalley 2008; Manzke et al. 2003; Montandon et al. 2011; Ren et al. 2009). In addition, other sites within the pontomedullary respiratory system including the rostral pons have been suggested as potential targets by some of these investigators. However, a recent study showed that extensive bilateral microinjections of the opioid antagonist naloxone (NAL) into the preBC region did not reverse the bradypnea induced by intravenous infusions of remifentanil (remi) at clinically relevant concentrations in decerebrate dogs (Mustapic et al. 2011). Moreover, direct microinjections of the μOR agonist [d-Ala2,N-Me-Phe4,Gly5-ol] enkephalin acetate (DAMGO) into the preBC region in decerebrate dogs resulted in tachypnea rather than bradypnea (Mustapic et al. 2011). Microinjections of the selective μOR agonist endomorphin-1 into the preBC region of anesthetized rats also produced a tachypneic response (Lonergan et al. 2003). Evidence from these in vivo studies suggests that the clinically observed μ-opioid-induced bradypnea occurs at sites outside of the preBC. There are other regions of the respiratory control system besides the preBC that exhibit high densities of μORs, and these may be more sensitive to systemic opioids than the preBC (Glatzer and Smith 2005; Martin-Schild et al. 1999). High densities of μOR immunoreactivity (μOR-ir) have been found in the parabrachial/Kölliker-Fuse (PB-KF) region of rats (Chamberlin et al. 1999; Ding et al. 1996) and cats (Sales et al. 1985). In fact, Hurle et al. (1985) found that μ- and δ-opioids applied to the dorsal surface of the pons near the PB region of cats produced a profound decrease in breathing rate with no change in tidal volume. In contrast, bilateral localized application to the ventral surface of the medulla resulted in a reduction in tidal volume and response to CO2 and an increase in breathing rate (Hurle et al. 1985). In addition, Eguchi et al. (1987) found that microinjections of morphine into the parabrachial nucleus (PBN) of anesthetized, vagotomized, paralyzed, and artificially ventilated cats produced a marked decrease in phrenic neurogram (PNG) burst rate. This evidence led us to perform exploratory experiments in decerebrate dogs as described below, which showed that pledgets saturated with DAMGO and applied to the dorsal surface of the pons between the inferior colliculi (IC) and superior cerebellar peduncles caused a profound bradypnea without any change in peak phrenic nerve activity. Consistent with those findings, immunohistochemical mapping of μORs in one dog showed high levels of μOR-ir in the region of the lateral PBN.
We thus hypothesized that clinically relevant concentrations of μOR agonists cause reduction in respiratory rate (bradypnea) via activation of pontine μORs. The purpose of the present studies was to more precisely define the pontine region in which μOR agonists produce bradypnea and to determine whether antagonism of μORs in that region reverses the bradypnea produced by systemic administration of clinically relevant concentrations of the μOR agonist remi.
METHODS
This research was approved by the subcommittee on animal studies of the Zablocki Department of Veterans Affairs (VA) Medical Center in accordance with provisions of the Animal Welfare Act, the Guide for the Care and Use of Laboratory Animals, and VA policy. Experiments were performed on 35 beagle dogs of either sex, weighing 9–12 kg. Inhalational anesthesia was induced by mask and maintained with isoflurane at 1.5–2.5% end-tidal concentration. The animals were monitored for signs of inadequate anesthesia such as salivation, lacrimation, and increases in blood pressure and heart rate. If required, anesthetic depth was increased immediately.
Surgical Procedures
Anesthesia was induced by mask with isoflurane, and the dogs were intubated with a cuffed endotracheal tube and their lungs mechanically ventilated with an air-O2-isoflurane mixture. The femoral artery was cannulated for blood pressure recording and blood gas sampling and the femoral vein for continuous infusion of maintenance fluids and administration of drugs. The animals were positioned in a stereotaxic device (model 1530; David Kopf Instruments, Tujunga, CA) with the head ventrally flexed (30°). A bilateral pneumothorax was performed to minimize brain stem movement and phasic inputs from chest wall mechanoreceptors. The animal was then decerebrated by midcollicular transection (Tonkovic-Capin et al. 1998), and isoflurane was discontinued. In a subset of dogs in protocol 2 (see below), studies were performed during isoflurane anesthesia without decerebration. The animals were ventilated with an air-O2 mixture and maintained in hyperoxic isocapnia (FiO2 >0.6, end-tidal CO2 range 40–50 mmHg). Complete access of the dorsal surface of the brain stem was obtained by an occipito-parietal craniotomy followed by cerebellectomy and external sagittal and nuchal bone crest removal. After bilateral neck dissections, phrenic nerve activity was recorded from the desheathed right C5 rootlet. Bilateral vagotomy was performed to achieve peripheral deafferentation to avoid interference of the mechanical ventilation with the underlying central respiratory rhythm and respiratory neuronal activity. The PNG was obtained from the moving-time average (100 ms) of the amplified phrenic nerve activity and was used to produce timing pulses corresponding to the beginning and end of the inspiratory phase for the measurement of inspiratory duration (TI) and expiratory duration (TE). Peak phrenic activity (PPA) was also obtained from the PNG. The breath-by-breath fictive breathing rate [phrenic activity burst rate; fB, breaths/min (BPM)] was calculated as 60/(TI + TE). Continuous neuromuscular block was achieved with pancuronium (0.1 mg·kg−1·h−1) to reduce motion artifacts during neuronal recordings. Esophageal temperature was maintained at 38.5 ± 1°C with a heating pad. If required, mean arterial pressure was maintained above 80 mmHg with adjustment of intravenous fluids and, when needed, phenylephrine infusion (0.5–5 μg·kg−1·min−1).
Microinjection Technique
A minimum of 1 h was allowed for preparation stabilization before data collection. A Hamilton microliter syringe (5 μl) with a calibrated scale (resolution: 50 nl) was used for microinjections of the μOR agonist DAMGO (100 μM) and the μOR antagonist NAL (100 μM) to locate μORs in the rostral pons that alter fB. The relatively high concentrations of DAMGO and NAL were used to saturate the μORs in a small region (∼1-mm diameter) of the pons to map out the extent of the opioid-sensitive region; remi was not used for injection at high concentrations since the only available formulation contains glycine, which is in itself inhibitory to neurons and would have thus confounded the results.
Remifentanil Infusion
The short-acting μOR agonist remi was continuously infused intravenously at a rate of ∼0.5 μg·kg−1·min−1, which is the analgesic dose that reduces the requirements of a volatile anesthetic to blunt surgical stimuli in humans and dogs by 50% (Michelsen and Hug 1996; Michelsen et al. 1996). This infusion rate was adjusted to produce >60% reduction in fB during hyperoxic isocapnea, which was maintained by mechanical ventilation.
Immunohistochemistry Methods Used for Preliminary Data
For immunohistochemical mapping of μOR, a carotid artery was cannulated rostrally in an anesthetized dog (n = 1) and perfused with phosphate-buffered saline (PBS) followed by 4% paraformaldehyde fixative with 0.2% picric acid in 0.1 M phosphate buffer. The brain was removed and postfixed in 4% paraformaldehyde fixative. Frozen transverse brain sections (25 μm) were cut from 10–17 mm rostral to the obex, and every fourth section was washed in PBS and incubated for 1 h in 5% normal goat serum in 0.3% Triton X-100 in PBS (PTX). Sections were then incubated in primary antibody, guinea pig anti-μOR-1 (1:3000 in 0.3% PTX; Millipore), for 24 h prior to Alexa Fluor 488-conjugated goat anti-guinea pig secondary (1:200; Invitrogen) for 1 h to yield a fluorescent signal for μOR. Sections were washed in PBS, floated onto slides, air-dried, dehydrated, and coverslipped. Control sections were processed simultaneously by incubation with serum instead of primary antibody and showed no staining for μOR. Sections were examined with a Nikon TE2000 microscope equipped for epifluorescence and photographed with an Optronics Quantifier 4 megapixel thermoelectrically cooled digital monochrome camera. Images were acquired at a resolution of 2,048 × 2,048 pixels with Optronics picture frame software and stored in TIFF format.
Preliminary Study Protocol: Dorsolateral Pons as a Potential Site of Opioid-Induced Bradypnea
Studies in four dogs, with experimental procedures similar to those described above, were used to examine the possible involvement of the dorsolateral pons in opioid-induced bradypnea. Pledgets saturated with 100 μM DAMGO or 500 μM and 10 mM NAL were bilaterally placed on the dorsal surface of the pons between the IC and the superior cerebellar peduncle. The pledgets consisted of strands, three per side, of ∼2-mm-diameter cotton string ∼10 mm in length. They were submerged in a beaker containing either DAMGO or NAL so as to be completely saturated. During the protocol, they were exchanged about every 6 min during a ∼30-min period to provide a continuous supply of the drug to the surface. After a maximum bradypneic response determined from the PNG, the dorsal pontine surface was rinsed with saline and NAL pledgets were then applied and exchanged as above to reverse the DAMGO-induced effect.
Protocols
Protocol 1: respiratory effects of direct pontine μOR activation via pontine microinjection of DAMGO.
In a first series of animals (n = 10), pontine microinjections of DAMGO were used to define the regions that mediate opioid-induced bradypnea. A 5-μl Hamilton syringe was stereotaxically positioned over the dorsal surface of the pons, between the IC and superior cerebellar peduncles. A series of injections of DAMGO (100 μM, 300–500 nl each), ∼10 min apart, were given covering 1 mm × 1 mm grids over a region from 4 to 8 mm lateral of midline and extending from 1 mm rostral to the caudal pole of the IC to 3 mm caudal to that pole at a depth of 3–4 mm from the dorsal surface (see Fig. 3, shaded areas). The 3- to 4-mm depth was suggested by results of preliminary studies. TI, TE, and PNG were continuously monitored online with a PowerLab system (ADInstruments, Castle Hill, Australia; 16SP and Chart). Average values of these variables and fB were obtained for off-line analysis from data starting 2 min before and for the 10-min period after each DAMGO microinjection. At the end of each experiment, bilateral microinjections (∼1 μl) of fluorescent latex microspheres (Lumafluor.com; Red Retrobeads), diluted to 5% of the supplied concentration, were used to mark the most sensitive areas for histology. The brain stems were removed postmortem and submersed in paraformaldehyde fixative (4%) for 1 wk before being frozen and sectioned. Sequential sections (25 μm) were cut from 10 to 17 mm rostral to obex. Alternate sections were stained with neutral red for histological identification of nuclear groups and compared with unstained adjacent sections showing fluorescent beads.

Schematic of the dorsal view of the canine pons/medulla with cerebellum removed showing the coordinate system used in this study. Shaded areas indicate the pontine region explored for opioid-induced bradypnea. The caudal pole of IC and the midline were used as the R-C and M-L initial (0/0) reference points, respectively.
Protocol 2: pontine microinjections of naloxone to reverse bradypnea induced by systemic remifentanil.
In a second series of animals (9 decerebrate and 12 anesthetized) after a control period of steady-state phrenic activity, remi was infused at an intravenous rate (0.1–1.0 μg·kg−1·min−1) to induce steady-state bradypnea (>60% decrease in central breathing frequency, fB) or apnea. During continued steady-state remi infusion, a series of unilateral and bilateral microinjections of NAL (100 μM, ∼550 nl each), within the opioid-sensitive pontine region delineated by the DAMGO protocol, were performed to localize the region in which it is possible to partially or totally antagonize the remi-induced bradypnea. Phrenic activity, TI, TE, and the NAL microinjection marker signals were recorded with a PowerLab system. After successful antagonism by the NAL microinjections, the intravenous remi infusion was discontinued and PNG activity was followed until full recovery occurred. Repeat runs in the same animal to allow for complete mapping of opioid reversal of the region were separated by ∼1 h to allow the microinjected NAL to diffuse out of the area. Microinjections of fluorescent beads were used to mark the most sensitive areas for histological purposes.
Data Analysis
Protocol 1: respiratory effects of direct pontine μOR activation via pontine microinjection of DAMGO.
The DAMGO microinjections resulted in a cumulative slowing of fB in sensitive areas. Therefore we calculated the incremental effect of each additional microinjection off-line. A new local control was obtained from the data ∼1 min prior to each microinjection. A second measurement was obtained 5–10 min after each microinjection but prior to the subsequent microinjection. These two values were used to calculate the percent change in fB. To aid in this analysis, the breath-by-breath data were fitted via a regression procedure known as LOESS or LOWESS (Cleveland and Devlin 1988), in which a series of subsets of the data is used to provide the best fit that follows the trend features of the data. SigmaPlot 11 (Systat Software, San Jose, CA) was used for this purpose and for plots used in the figures. Examples of the fits are indicated with red lines in the fB data (BPM) in Fig. 1, A and B. Figure 1A shows an example of the effects of a series of DAMGO microinjections (vertical arrows) at the three locations indicated on breath-by-breath fB (BPM) with the data fit superimposed (red line). Note the cumulative effect of the microinjections on the slowing of phrenic respiratory rate. Time-expanded plots of the same data for each microinjection site are shown with time shifted relative to the time of microinjection in Fig. 1B (black arrowheads at microinjection, t = 0). From these plots, the incremental changes in fB were calculated as a percent change from the preinjection local control values as indicated by arrows in Fig. 1B. Contour plots were constructed to display the percent change in fB as a function of the location on the rostral-caudal (R-C) by medial-lateral (M-L) grid (Fig. 1A, bottom).

Example of data illustrating the methods used to quantify the effects of [d-Ala2,N-Me-Phe4,Gly-ol5]-enkephalin (DAMGO) microinjections during the pontine mapping protocol (protocol 1). Microinjections of DAMGO (100 μM, 500 nl) were sequentially injected beginning at 0 rostral-caudal (R-C) and 4 lateral and progressed laterally until 8 mm lateral. The medial to lateral sequence of microinjections was subsequently repeated for −1, −2, −3, +1 R-C. Typical depth of injections was 3 mm from dorsal surface (see 3rd coordinate). A, top: effects of a series of DAMGO microinjections [vertical arrows with coordinates as indicated: R-C/medial-lateral (M-L)/depth (D)] on the phrenic neurogram (PNG) and breath-by breath values of TI (blue symbols), TE (red symbols) and fictive breathing rate [fB, breaths/min (BPM), black symbols]. The corresponding locations are shown by the 3 black circles at 0 R-C on the contour plot (A, bottom). Thick red line in rate data is the local regression curve (LOWESS function) indicating the best-fit trend. Note that there is a progressive increase in TI and TE, a decrease in rate, and no change in peak PNG. B: time-expanded plots of fB for the corresponding microinjections indicated on the contour plot (black circles) with time of injection set to 0 (vertical dashed lines). Red lines: best-fit regression curves. Because of cumulative effects of DAMGO, incremental % changes relative to the preinjection values (dashed horizontal lines) were calculated 6–10 min after injection (arrows with values). Arrowheads: time of microinjections. R-C, lateral, and depth coordinates are given at right of each plot. Contour plot (A, bottom) shows % reduction in breathing rate caused with DAMGO microinjections at the various R-C and M-L locations. In this example, the maximum change in fB of ∼25% occurred at −2 R-C and 6 lateral. Corresponding data are shown in B, bottom, with changed scale.
Protocol 2: pontine microinjections of naloxone to reverse bradypnea induced by systemic remifentanil.
Breath-by-breath values of TI, TE, fB, and PPA were computed off-line continuously for the pre-remi baseline period, during intravenous remi infusion, and the post-remi infusion period. The LOWESS function was used to smooth the fB data from which values were obtained to calculate the amount of remi-induced depression and the amount of reversal of that depression for each NAL microinjection. The following expressions were used to calculate the drug effects:
where fcon is pre-remi control fB, fremi is fB during steady-state remi-induced bradypnea, f(i)NAL is the value of fB 5–10 min after the ith NAL injection, and i designates the injection number within a sequence of microinjections during a remi protocol. The number of NAL injections is determined by the number required to obtain complete reversal of the remi effect.
Statistical Analyses
A one-way ANOVA was used to analyze changes in fB due to microinjections at locations that were responsive to either DAMGO or NAL. The Holm-Sidak method was used for pairwise multiple comparisons with a family-wide error rate of 0.05. For all data sets, tests for normality (Kolmogorov-Smirnov test) were performed before parametric procedures were used. For data sets that failed the normality test, a Kruskal-Wallis one-way ANOVA on ranks was used with Dunn's method for multiple comparisons versus control group. Differences were considered significant at P < 0.05. Values are expressed as means ± SE.
RESULTS
Preliminary Study Findings
An example of the preliminary data showing the effects of 100 μM DAMGO applied to the dorsal surface of the pons between the IC and the superior cerebellar peduncle is shown in Fig. 2A. The PNG burst rate decreased from ∼54 BPM to ∼4 BPM (∼92% reduction). As TI increased, the peak PNG also increased slightly. At ∼60 min into the protocol, the dorsal surface was rinsed with warm saline, and NAL (500 μM) pledgets, which were refreshed every ∼6 min for an hour, were placed bilaterally to antagonize the DAMGO effects. This was followed by replacing the 500 μM NAL with 10 mM NAL to obtain a more complete reversal. For the group, there was a 51 ± 15% reduction in PNG burst rate (n = 4) by the dorsal surface application of μ-opioids. In addition, immunohistochemical mapping of μORs in one dog showed high levels of μOR-ir in the region of the lateral PBN (Fig. 2B). Together, these findings led us to hypothesize that systemically administered μ-opioids produce bradypnea via μORs in the dorsolateral rostral pons.

Preliminary data suggesting that μ-opioid receptors (μORs) in the dorsolateral pons may be the targets of systemically administered opioids that produce bradypnea at clinically relevant concentrations. A: example of the effects of DAMGO-saturated pledgets applied to the dorsal surface of the pons between the inferior colliculus (IC) and the superior cerebellar peduncle (CP) of a dog on PNG and fB. Note marked bradypnea and increases in TI and TE, with a small increase in peak PNG. Naloxone (NAL)-saturated pledgets gradually reversed the DAMGO-induced bradypnea. B: distribution of μOR immunoreactivity (μOR-ir) in the rostral dorsal lateral pons showing the parabrachial/Kölliker-Fuse (PB-KF) complex. Center: fluorescence photomicrograph of a coronal section that shows a relatively high level of μOR-ir lateral to the superior cerebellar peduncle (arrowheads a–c) corresponding to the lateral PB complex. a and c: Higher-magnification images near arrowheads a and c show discrete points of high-density μOR-ir on cell somas. b: High level of μOR-ir of a filamentous network, possibly dendritic, near arrowheads b.
Protocol 1: Respiratory Effects of Direct Pontine μOR Activation via Pontine Microinjection of DAMGO
In 10 dogs, 286 DAMGO microinjections (390 ± 13 nl/microinjection; 28.6 ± 3.3 microinjections/dog) in the dorsal lateral pons extending from 1 mm rostral to the caudal pole of the IC to 3 mm caudal and from 4 to 8 mm lateral of the midline (shaded areas in Fig. 3) were performed to delineate the region that mediates an opioid induced decrease in fB. Figure 4 shows an example of a series of unilateral DAMGO (100 μM, 500 nl each) microinjections in the lateral direction, which resulted in significant slowing from ∼11 BPM to apnea (Fig. 4, bottom, fB). The ∼10-min apnea was subsequently reversed by intravenous NAL (∼10 μg/kg; Fig. 4, right). PPA increased with the increase in TI. Time-expanded records of PNG (Fig. 4, top) are shown in Fig. 5A and superimposed traces of the first cycle in each record in Fig. 5B. Note that the ramp slope of the PNG is not altered and the peak height is dependent on magnitude of TI, suggesting that respiratory drive has not changed. A nonlinear relationship between TI and the following TE of each cycle is also seen after vagotomy (Fig. 5C). While DAMGO increases both TI and TE, the major decrease in fB is primarily due to the increase in TE.

Example of the effects of a series of unilateral DAMGO microinjections (diamond symbols with pontine R-C/lateral coordinates). Note the progressive increases in TI and TE and decrease in fB and subsequent apnea. An IV bolus of NAL (10 μg/kg) partially antagonized the apnea. Larger subsequent doses were required to fully antagonize the DAMGO effects. A moderate increase in peak PNG is entirely due to the increase in TI without changes in drive (see Fig. 5 and text for further explanation).

Pontine DAMGO microinjections produce changes in timing but not drive. A: time-expanded segments of PNG record in Fig. 4, top, at the times indicated (a–e) below the PNG trace. The 1st PNG cycle in each segment has been time aligned at 0 s. B: superimposed traces of the 1st breath cycle in each record. Note that the slope of the PNG ramp is not altered and the peak height is dependent on magnitude of TI, suggesting that respiratory drive has not changed. C: plot of TI vs. the subsequent TE of each cycle during vagotomy appears to be nonlinear. While DAMGO caused increases in both TI and TE, the large decreases in fB are mainly due to the large increases in TE.
The data of the contour plots from 10 dogs were averaged at each of the grid coordinates to produce the contour plot of the average responses (Fig. 6, top). The average magnitude of the incremental responses in fB for the most sensitive locations, indicated by superimposed open circles in Fig. 6, top, are shown in Fig. 7A. The largest incremental responses were located at 5- to 6 mm lateral to midline and 0 to −1 caudal to the IC and were in the range of 15–20%. The cumulative maximum effect of a series of DAMGO microinjections per experiment was on average a 74 ± 6% reduction in baseline fB from 18.6 ± 4.2 to 5.0 ± 1.5 BPM with 8.2 ± 1.1 microinjections. PPA was not significantly changed (0.78 ± 1.52%, n = 74 microinjections) by the DAMGO microinjections. The prominent and most consistent response over the lateral range of 4 to 8 mm from the midline was a >5% decrease in fB as shown by the pooled response data from all R-C sites corresponding to each lateral site (Fig. 7B, Bradypnea). At some of the sites in some animals, DAMGO microinjections caused no response or an increase in fB. However, increases in fB >5% were seldom seen at 5–7 mm lateral (Fig. 7B, Tachypnea) and were observed less often than little or no responses (Fig. 7B, No Response).
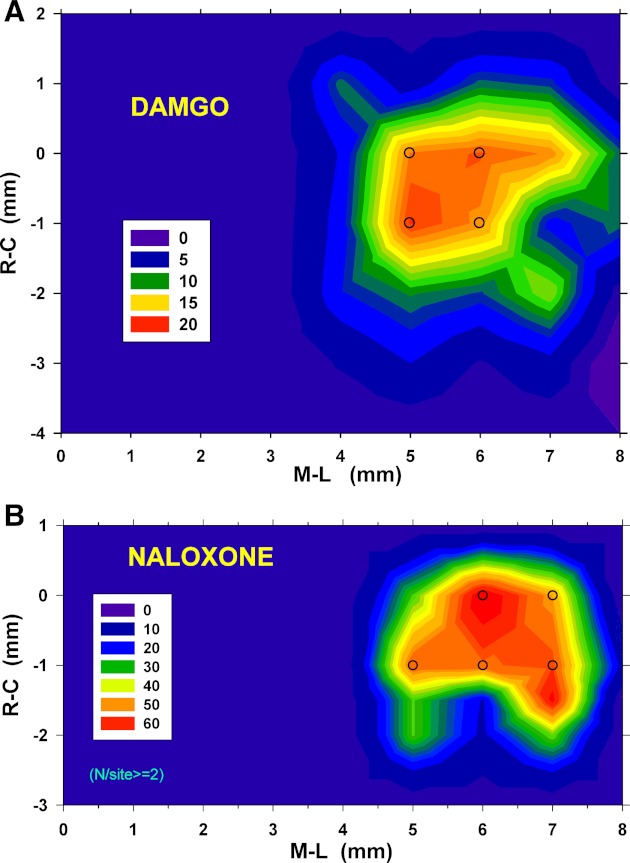
Top: contour plot for DAMGO-induced decreases in breathing frequency based on mean % change in fB values obtained in 10 dogs. The most opioid-responsive region was confined to an area of <2 × 2 mm. The largest decreases in fB at any injection site were ∼20% and were located at −1 R-C and 5 lateral. Superimposed open circles indicate sites from which data were tested for statistical significance (see text for details) (Fig. 7A). Bottom: contour plot for NAL microinjection reversal of IV remifentanil (remi)-induced bradypnea. Values are the average % reversal of the maximum depression of fB caused by remi from 21 dogs (see methods for more details regarding calculations). The largest mean values were ∼60% (red). Open circles indicate sites where data were statistically analyzed as shown in Fig. 7C.

A: % change in fB for each microinjection of 300–500 nl of 100 μM DAMGO at the site indicated above each bar (also in Fig. 6A, open circles; n = 10 dogs). #P < 0.05 difference from zero change (1-way ANOVA). B: types of responses to DAMGO as % of the number (N) of DAMGO microinjections per lateral coordinate (all R-C locations pooled). Tachypnea was rarely observed throughout the mapped region (darkest bars). C: % reversal of the remi-induced depression of fB per NAL microinjection (540 nl of 100 μM NAL). *P < 0.05, **P < 0.01 changes relative to no effect (1-way ANOVA).
Protocol 2: Pontine Microinjections of Naloxone to Reverse Bradypnea Induced by Systemic Remifentanil
The reversal of remi-induced bradypnea by NAL microinjections in the region delineated by the DAMGO microinjections (protocol 1) was studied in 21 dogs. An example of a protocol is given in Fig. 8, where remi (0.8 μg·kg−1·min−1) markedly decreased the peak PNG and PNG ramp slope (not shown) and altered fB. As illustrated in this example, after initiation of the remi infusion a transient increase in respiratory rate (tachypnea) was frequently observed (e.g., fB above the dashed horizontal line, Fig. 8). This was followed by bradypnea and finally apnea as the remi infusion continued. Throughout this time, PPA gradually decreased until it reached a plateau well above zero (see PNG record before start of NAL microinjections and after and time-expanded records c–f, Fig. 8). This suggests that the apnea resulted from an interruption of respiratory rhythm rather than a completely suppressed peak PNG amplitude. The intermittent large tidal breaths illustrated in the peak PNGs are sighlike cycles that are also frequently observed during remi infusions. During the apneic period, short periods of phrenic activity reappeared, suggesting that the selected remi infusion rate did not depress respiratory activity much below the phrenic apneic threshold. After ∼10 min of apnea, bilateral pontine microinjections of NAL (1 μl each) were made at 7 mm lateral to the midline and 1 mm caudal to the caudal pole of the IC at a depth of ∼3 mm, which completely reversed the apnea and resulted in a transient respiratory rate above the pre-remi control frequency (transient tachypnea). In this example, the first NAL microinjection was sufficient to initiate the reversal of the apnea. Despite these profound effects of pontine NAL microinjections on fB, there was very little effect on peak PNG (Fig. 8, middle section between the 2 arrows). As the NAL effect gradually declined during continued remi infusion, bradypnea and apnea reoccurred. After termination of the remi infusion, peak PNG and fB gradually returned to pre-remi levels. The length of the entire protocol was almost 2 h.

Example of the reversal of IV remi-induced bradypnea/apnea by NAL (100 μM) microinjections in the dorsolateral pons (−1 mm R-C, 7 mm lateral to midline, 3 mm ventral to dorsal surface). The IV remi infusion at a rate of 0.8 μg·kg−1·min−1 decreased peak PNG, PNG slope, and breathing rate fB until transient apneas ensued (PNG record, arrows). Intermittently, large sighlike respiratory cycles were also observed. A transient increase in fB above control baseline (RATE record, horizontal dashed line) was observed after initiation of remi infusion prior to bradypnea. Throughout the infusion, peak PNG gradually decreased until a steady-state plateau at ∼20% peak PNG was reached. Peak PNG remained depressed after the two bilaterally symmetrical 1-μl NAL microinjections (compare records at times c, d, e, and f), while respiratory rate transiently recovered despite ongoing remi infusion. During the initial apneic period, short periods of phrenic activity reappeared (between times c and e), suggesting that the opioid plasma concentration was close to the phrenic apneic threshold. After 10 min of apnea, the first unilateral NAL microinjection reversed the apnea (the 2nd contralateral microinjection given as part of the protocol), and a transient tachypnea similar to the initial one resulted without any notable change in peak PNG. As the effect of the bilaterally microinjected NAL gradually declined during sustained IV remi infusion, bradypnea and apnea eventually reoccurred. After termination of the remi infusion, both peak PNG and fB gradually returned to pre-remi levels. The entire protocol lasted almost 2 h. Time-expanded records of PNG are shown for times indicated by the labeled arrows (a–g). e: Onset of reversal of the apnea by the 1st NAL microinjection, where the 1st PNG cycle shows a sigh in this case.
During 39 NAL microinjection protocols, the pre-remi fB of 14.8 ± 1.6 BPM was reduced by 72 ± 4% by 0.41 ± 0.05 μg·kg−1·min−1 of intravenous remi. The level of remi-induced depression of fB was similar in decerebrate (n = 18 runs) versus isoflurane-anesthetized (n = 21 runs) dogs (−64 ± 6% vs. −78. ± 3%); however, to obtain this same level of depression, decerebrate dogs required a ∼35% higher remi infusion rate than anesthetized dogs (0.46 ± 0.04 vs. 0.34 ± 0.04 μg·min−1·kg−1; P = 0.029). Remi also reduced the peak PNG by 25.3 ± 3.6%. There was no significant correlation between the degree of remi-induced depression in peak PNG and fB (r = −0.24; P = 0.14, Spearman rank order).
A total of 84 NAL microinjections (540 ± 35 nl each) in 21 dogs (decerebrate and anesthetized) were used to map the region where reversal of remi-induced bradypnea was observed (Fig. 6, bottom). The average magnitude of the pooled responses at the most sensitive NAL locations of Fig. 6, bottom (open circles) are given in Fig. 7C. The magnitude of bradypnea reversal was ∼50% per NAL microinjection, and typically two NAL microinjections in these locations were able to completely reverse remi-induced bradypnea or apnea (101.8 ± 11.3%). There was no difference in the amount of NAL-induced reversal of the remi effect between the two types of preparations (107 ± 9% vs. 82 ± 14%, respectively). A total of 59 of the 84 NAL microinjections were located within the sensitive region extending from 0 to −1 mm rostrally and from 5 to 7 mm laterally (Fig. 7C). Unilateral microinjections (28/59) were just as effective as the bilateral microinjections (31/59), 46.7 ± 9.5% vs. 49.8 ± 8.2% (P = 0.60) reversal/microinjection. For 12 protocols in 9 of the dogs, only a single microinjection at the first site selected within the sensitive region was able to produce complete reversal. The NAL microinjections that reversed remi-induced bradypnea had no effect on peak PNG (0.75 ± 4.1% change; P = 0.86) and only a small increase (7.1 ± 2.5% change; P = 0.02) in PNG/TI (average slope of the PNG).
Since the remi-induced bradypnea protocols take 1.5–2 h to complete and only a small number of NAL microinjections were required to totally reverse the bradypnea, the number of NAL microinjections per experiment was less than the number of DAMGO injections in protocol 1.
Histological Identification of Most Effective NAL Injection Sites
Microinjections of fluorescent beads at the end of an experiment were placed bilaterally in the NAL microinjection sites that had produced the largest responses in fB. An example of the location of the fluorescence in the PB region of the dorsolateral pons is shown in Fig. 9, left; the open circle indicates the location of the tip of the electrode at the bottom of the track that has been filled by the beads (arrow) during injection. The diagram in Fig. 9, right, shows the most effective sites for NAL reversal of remi-induced bradypnea, where the asterisks indicate sites based on the histologically verified fluorescent bead microinjections and the open circles indicate sites based on stereotaxic coordinates relative to the midline and depth. NAL microinjections in and near the brachium pontis (Fig. 9, right, asterisks) resulted in nearly complete (78–100%) reversal of the remi-induced bradypnea. Both the histological and the stereotaxic data from the NAL microinjection protocol suggest that activation of μORs in the PB-KF complex at a depth of 3–4 mm ventral to the dorsal surface mediates the intravenous remi-induced bradypnea.

Left: section of rostral pons at the level of the caudal pole of the inferior colliculus (0 R-C) indicating the location of a microinjection in the most sensitive response area in this dog. The open circle indicates the location of the tip of the electrode at the bottom of the track (0 mm R-C, 6 mm lateral, 4.2 mm deep) that has been filled by the fluorescent beads (arrow) during injection and is just medial to KF. Right: diagram shows the most effective sites for NAL reversal of IV remi-induced bradypnea, where asterisks indicate sites based on the fluorescent bead microinjections and open circles indicate sites based on stereotaxic coordinates relative to the midline and depth for R-C coordinates from 0 to −2 mm relative to the caudal pole of the IC. NAL microinjections in and near the brachium pontis (lateral-most asterisks) resulted in nearly complete (78–100%) reversal of the remi-induced bradypnea, suggesting that μORs in the lateral parabrachial-KF complex mediate the remi-induced bradypnea. DTN, dorsal tegmental nucleus; KF, Kölliker-Fuse nucleus; LC, locus coeruleus; LLV, lateral lemniscus ventral nucleus; LPB, lateral parabrachial nucleus; MLB, medial longitudinal bundle; MPB, medial parabrachial nucleus; PG, pontine gray; SCN, superior central nucleus; SCP, superior cerebellar peduncle; TF, tegmental field.
DISCUSSION
This study shows that μORs in the pontine PB region play a key role in the control of breathing frequency in an adult in vivo preparation. In addition, this is the first study to show that μORs in this region are the primary targets where systemically administered μOR agonists produce bradypnea. Mapping with DAMGO microinjections delineated a 2 mm × 2 mm area, extending from ∼5 to 7 mm lateral of midline and from the caudal pole of the IC to 2 mm caudal of IC, in which it was possible to produce bradypnea in midcollicular decerebrate dogs. Within this same region, microinjections of NAL partially or totally reversed the depression of respiratory rate induced by intravenous remi in a clinically analgesic dose range in both decerebrate and anesthetized dogs.
In the DAMGO protocol, ∼400-nl microinjections were made 1 mm apart in a gridlike manner. The estimated effective spherical radius of the microinjected drug, taking into account an extracellular volume fraction of 0.21 (Nicholson 1985) and without subsequent diffusion (i.e., the initial volume), is ∼750 μm. This provides overlapping volumes for microinjections spaced 1 mm apart. Since the brain stem with the cerebellum removed allowed direct visualization of the pons, stereotaxic measurements relative to the midline and caudal pole of the IC could be accurately and consistently made. Because DAMGO microinjections in this region produced a cumulative effect, it was necessary to estimate the incremental change in fB for each subsequent microinjection as described in methods. The largest changes in fB per microinjection ranged from 15% to 20% (Fig. 6, top, and Fig. 7A). Since PPA on average was not affected, we conclude that the μORs in this pontine region alter respiratory phase timing rather than respiratory drive, and this primarily by increases in TE (e.g., Figs. 1A, ,4,4, and and55).
We found that DAMGO microinjections did not reduce peak PNG; however, intravenous remi reduced the peak PNG on average by 25.3 ± 3.6%. This suggests that remi may have been acting at multiple sites within the respiratory control system, possibly in the ventral medulla as suggested by the study of Hurle et al. (1985) in spontaneously breathing, anesthetized cats.
The main objective of our second protocol was to determine whether the μORs that were activated by DAMGO and caused bradypnea in the first protocol were also responsible for the bradypnea produced by intravenous remi at clinical tissue concentrations. Remi was used in our study because of its rapid onset and short latency to peak effect, as well as its rapid recovery (context-sensitive half-life of ∼4 min; Burkle et al. 1996), which is independent of dose rate and length of infusion and allowed us to perform multiple exposures in the same experimental animal. The dose rate that abolishes reaction to surgical stimuli in 50% of patients was found to be ∼0.5 μg·kg−1·min−1 and appears to be similar in dogs (Michelsen and Hug 1996; Michelsen et al. 1996).
Larger microinjection volumes for NAL (540 ± 35 nl each), confined to the region delineated by the DAMGO protocol, reached a greater number of receptors per microinjection (effective spherical radius ∼1 mm), achieving a larger reversal effect per injection (∼50% reversal) and thus shortening each remi infusion protocol. Typically, two or three remi infusion protocols with stable fictive breathing patterns were possible per experiment. Interestingly, symmetrical bilateral microinjections were no more effective than unilateral microinjections, even though twice as much volume was injected. The reason for this is not clear.
Role of Pontine Parabrachial/Kölliker-Fuse Complex in Control of Breathing Frequency
The results of this study emphasize the powerful role that the pontine PB-KF complex plays in the control of phase timing and breathing frequency (e.g., Fig. 4). The range of fB control is remarkable, since microinjected DAMGO was capable of producing significant bradypnea up to complete respiratory arrest through increases in TE. On the other hand, remi-induced bradypnea of similar magnitude (∼72% reduction in baseline fB) could be fully reversed by the pontine NAL microinjections.
The importance of the pontine PB-KF complex in the control of breathing has been known for some time. This area has been referred to as the “pneumotaxic center” or, more recently, the pontine respiratory group (PRG) (Bianchi et al. 1995; Feldman 1986). PRG neurons project to the ventral respiratory group (Chamberlin et al. 1999). Electrical stimulation or chemical stimulation with glutamate in the lateral PB complex in cats or rats predominantly evoked respiratory facilitation, while stimulation of the more ventrally located Kölliker-Fuse nucleus or medial PBN decreased respiratory rate (Chamberlin and Saper 1994; Cohen 1971; Lara et al. 1994). Song and Poon (2009) also showed that bilateral lesions of the lateral PBN resulted in significant bradypnea (increase in TE) under hyperoxia and in marked attenuation of the tachypneic response (decrease in TE) to hypoxia. In vitro voltage-clamp experiments in rat lateral PB neurons show that μOR agonists increase the potassium conductance of the membrane and inhibit the neurons through hyperpolarization (Christie and North 1988). It is thus reasonable to deduce that the inhibition of neurons whose excitation results in tachypnea would cause bradypnea.
The maximum responses to DAMGO and NAL microinjections were located 5–7 mm lateral from midline, 0–1 mm caudal of the inferior colliculi, and 3–4 mm deep and correspond histologically with the structures of the PBN. There were more tachypneic responses medial of this area, suggesting that DAMGO microinjections into the medial PBN may have inhibited neurons that produce bradypnea (Lara et al. 1994). Still, in a few animals DAMGO microinjections into the presumed lateral PBN had no effect or resulted in tachypnea (Fig. 7B), possibly due to anatomical differences among animals. The fact that complete reversals of bradypnea by single NAL microinjections were seen in the most lateral locations (i.e., 7 mm lateral, Fig. 6, bottom; also example in Fig. 8) suggests that the lateral PB region may play a significant role in the bradypneic effect of clinically used μOR agonists. However, significant effects were also found at 6 mm lateral to midline, which appears to correspond with the medial PBN. Because of the size of the microinjections and the subsequent diffusion, our ability to resolve the location within the PB complex is limited.
Other Brain Stem Sites Possibly Involved with Opioid-Induced Respiratory Depression
In adult rats, small numbers of weakly μOR-ir neurons and more abundant μOR-ir fibers and terminals were found throughout the rostrocaudal extent of the VRG (Lonergan et al. 2003). Interestingly, there appeared to be no difference in the number of μOR-ir neurons in the preBC compared with the Bötzinger complex. Other respiratory-related areas with intense μOR-like immunoreactivity were the pontine lateral PBN, the locus coeruleus, the rostral ambiguus nucleus, and the medial and commissural subnuclei of the NTS (Chamberlin et al. 1999; Ding et al. 1996). The mere presence of μORs, however, does not allow any conclusions as to their clinical significance. Similarly, the presence of μ- and δ-opioid receptors on canine respiratory premotor neurons in the VRC, demonstrated by picoejection of the respective agonist onto single neurons, did not translate into a depression of these neurons by clinical concentrations of a μOR agonist (Stucke et al. 2008). The apparent reason was that the concentrations required for neuronal depression were in the 100–1,000 μM range with a threshold of 10 μM for morphine (Stucke et al. 2008). Perhaps the concentration of synaptically released endogenous opioids is high enough to be effective on these neurons. In contrast, the plasma concentration of remi in dogs at the infusion rate used in the present study (∼0.5 μg·kg−1·min−1) is estimated to be ∼14 nM (Michelsen et al. 1996), and the effect site concentration (extracellular fluid) may be ∼50% lower (Kabbaj et al. 2005). In whole brain tissue, the binding affinity for remi was found to be 21.1 nM (Poisnel et al. 2006).
NAL microinjections did not always achieve a full reversal of the remi effect. Although this could be due to the spread of the drug within the PBN, it is possible that other areas of the brain stem may also contribute, to a lesser degree, to the opioid-induced respiratory depression. With microdialysis of fentanyl (100 μM) into the preBC region of anesthetized spontaneously breathing adult rats, it was reported that the preBC was solely responsible for the respiratory rate suppression by systemic opioids (Montandon et al. 2011). However, this study used relatively large, stereotaxically guided (relative to bregma) microdialysis probes (0.24-mm diameter, 1-mm length of permeable membrane) located at an average distance of 760 μm from the preBC (n = 8; based on corrected vector differences from Supplemental Table 1 in Montandon et al. 2011). Since the respiratory effect was measured after dialyzing 100 μM fentanyl over 30–60 min, it is very difficult to judge the amount of fentanyl released or its concentration distribution within the brain stem. For example, genioglossus muscle activity was significantly depressed, even though the presumed location of hypoglossal premotor neurons was on average twice as far from the dialysis probes as the preBC (Supplemental Table 1 in Montandon et al. 2011). The effective concentration of fentanyl in the brain stem most likely exceeded by far the plasma concentration of ∼24 nM, which has been shown to depress minute ventilation by 57% in awake adult rats (Yassen et al. 2005). Montandon et al. (2011) used an indirect method based on probe locations and response latencies to estimate the site of respiratory depression. However, because of the heterogeneity of brain tissue, the rate of diffusion will vary with path direction, as shown in their data by comparing diffusion-front velocities (distance/latency; Supplemental Table 1 in Montandon et al. 2011), and result in dubious estimates of effect-site locations.
Other in vivo studies suggest that the preBC is not the location of opioid-induced respiratory depression. Unilateral microinjection of the potent endogenous μOR agonist endomorphin-1 (60 nl, 10 mM, binding affinity Ki: 0.36 nM; Zadina et al. 1997) into both the preBC and Bötzinger complex in adult rats produced an increase in phrenic burst rate, rather than a bradypneic response that is observed clinically (Lonergan et al. 2003). These findings are consistent with those in decerebrate dogs, where localized microinjections of DAMGO (∼140 nl, 100 μM) within the functionally identified preBC region produced tachypnea (44% increase in fB; n = 16), while intravenous infusion of remi counteracted the DAMGO-induced tachypnea and produced marked bradypnea (55% decrease in fB relative to baseline control; Mustapic et al. 2010). More importantly, multiple bilateral microinjections of NAL (500 μM, 120 nl each, 3 per side) into the preBC region during steady-state, remi-induced bradypnea had no effect on fB and PPA (Mustapic et al. 2010). Finally, in awake goats, relatively large microinjections (10 μl) of DAMGO (100 nM) into the preBC had no effect on the eupneic breathing pattern (Krause et al. 2009). Altogether, these studies suggest that the preBC is not directly involved in decrease in respiratory rate by clinical concentrations of opioids.
Because of the invasive nature of our studies that require anesthesia, it is not possible to assess the opioid-induced effects on breathing in the cortex and other supra-brain stem structures, which may play an important role in the awake state. For example, Pattinson et al. (2009), using MRI studies in awake volunteers, showed that remi reduced the urge to breathe and this was associated with a decrease in activity in the left anterior insula and operculum cortical areas. However, our studies were focused on the effects of opioids on the automatic control of breathing, where opioids cause dose-dependent bradypnea.
Summary
We have shown that microinjections of DAMGO in the region of the PBN cause significant decreases in fB and microinjections of NAL in the same PB region completely reverse the bradypnea induced by systemically administered opioids at concentrations clinically relevant for analgesia. Thus it appears that the PB complex, which is a major relay center for converging visceral, nociceptive, and thermoreceptive information to the forebrain (Benarroch 2006; Jiang et al. 2004; Saper 2002), is a major site of opioid-induced respiratory depression.
GRANTS
Support for this work was received from the Department of Anesthesiology, Medical College of Wisconsin, Children's Hospital of Wisconsin, and the Zablocki VA Medical Center. This work was supported by VA Medical Research Funds (Washington, DC) (to E. J. Zuperku) and National Institute of General Medical Sciences Grant GM-59234-06A1 “Volatile Anesthetics and Respiratory Neurotransmission” (to E. A. E. Stuth).
DISCLOSURES
No conflicts of interest, financial or otherwise, are declared by the author(s).
AUTHOR CONTRIBUTIONS
Author contributions: I.P., S.M., and T.R. performed experiments; I.P., S.M., T.R., and E.J.Z. analyzed data; I.P., A.G.S., E.A.E.S., F.A.H., C.D., and E.J.Z. edited and revised manuscript; I.P., A.G.S., E.A.E.S., and E.J.Z. approved final version of manuscript; A.G.S., E.A.E.S., and E.J.Z. conception and design of research; A.G.S., E.A.E.S., F.A.H., C.D., and E.J.Z. interpreted results of experiments; E.J.Z. prepared figures; E.J.Z. drafted manuscript.
ACKNOWLEDGMENTS
The authors thank Jack Tomlinson, Biological Laboratory Technician, Zablocki VA Medical Center, for excellent technical assistance.
REFERENCES
- Benarroch EE. Pain-autonomic interactions. Neurol Sci 27, Suppl 2: S130–S133, 2006 [PubMed] [Google Scholar]
- Bianchi AL, Denavit-Saubie M, Champagnat J. Central control of breathing in mammals: neuronal circuitry, membrane properties, and neurotransmitters. Physiol Rev 75: 1–45, 1995 [PubMed] [Google Scholar]
- Burkle H, Dunbar S, Van Aken H. Remifentanil: a novel, short-acting, mu-opioid. Anesth Analg 83: 646–651, 1996 [PubMed] [Google Scholar]
- Chamberlin NL, Mansour A, Watson SJ, Saper CB. Localization of mu-opioid receptors on amygdaloid projection neurons in the parabrachial nucleus of the rat. Brain Res 827: 198–204, 1999 [PubMed] [Google Scholar]
- Chamberlin NL, Saper CB. Topographic organization of respiratory responses to glutamate microstimulation of the parabrachial nucleus in the rat. J Neurosci 14: 6500–6510, 1994 [PMC free article] [PubMed] [Google Scholar]
- Christie MJ, North RA. Agonists at mu-opioid, M2-muscarinic and GABAB-receptors increase the same potassium conductance in rat lateral parabrachial neurones. Br J Pharmacol 95: 896–902, 1988 [PMC free article] [PubMed] [Google Scholar]
- Cleveland WS, Devlin SJ. Locally weighted regression: an approach to regression analysis by local fitting. J Am Stat Assoc 83: 596–610, 1988 [Google Scholar]
- Cohen MI. Switching of the respiratory phases and evoked phrenic responses produced by rostral pontine electrical stimulation. J Physiol 217: 133–158, 1971 [PMC free article] [PubMed] [Google Scholar]
- Ding YQ, Kaneko T, Nomura S, Mizuno N. Immunohistochemical localization of mu-opioid receptors in the central nervous system of the rat. J Comp Neurol 367: 375–402, 1996 [PubMed] [Google Scholar]
- Eguchi K, Tadaki E, Simbulan D, Jr, Kumazawa T. Respiratory depression caused by either morphine microinjection or repetitive electrical stimulation in the region of the nucleus parabrachialis of cats. Pflügers Arch 409: 367–373, 1987 [PubMed] [Google Scholar]
- Feldman JL. Neurophysiology of breathing in mammals. Handbook of Physiology. The Nervous System. Intrinsic Regulatory Systems of the Brain. Bethesda, MD: Am Physiol Soc, 1986, sect. 1, vol. IV, p. 463–524 [Google Scholar]
- Glatzer NR, Smith BN. Modulation of synaptic transmission in the rat nucleus of the solitary tract by endomorphin-1. J Neurophysiol 93: 2530–2540, 2005 [PubMed] [Google Scholar]
- Gray PA, Rekling JC, Bocchiaro CM, Feldman JL. Modulation of respiratory frequency by peptidergic input to rhythmogenic neurons in the preBötzinger complex. Science 286: 1566–1568, 1999 [PMC free article] [PubMed] [Google Scholar]
- Haji A, Yamazaki H, Ohi Y, Takeda R. Distribution of mu receptors in the ventral respiratory group neurons; immunohistochemical and pharmacological studies in decerebrate cats. Neurosci Lett 351: 37–40, 2003 [PubMed] [Google Scholar]
- Hurle MA, Mediavilla A, Florez J. Differential respiratory patterns induced by opioids applied to the ventral medullary and dorsal pontine surfaces of cats. Neuropharmacology 24: 597–606, 1985 [PubMed] [Google Scholar]
- Janczewski WA, Onimaru H, Homma I, Feldman JL. Opioid-resistant respiratory pathway from the preinspiratory neurones to abdominal muscles: in vivo and in vitro study in the newborn rat. J Physiol 545.3: 1017–1026, 2002 [PMC free article] [PubMed] [Google Scholar]
- Jiang M, Alheid GF, Calandriello T, McCrimmon DR. Parabrachial-lateral pontine neurons link nociception and breathing. Respir Physiol Neurobiol 143: 215–233, 2004 [PubMed] [Google Scholar]
- Kabbaj M, Vachon P, Varin F. Impact of peripheral elimination on the concentration-effect relationship of remifentanil in anaesthetized dogs. Br J Anaesth 94: 357–365, 2005 [PubMed] [Google Scholar]
- Krause KL, Neumueller SE, Marshall BD, Kiner T, Bonis JM, Pan LG, Qian B, Forster HV. Micro-opioid receptor agonist injections into the presumed pre-Bötzinger complex and the surrounding region of awake goats do not alter eupneic breathing. J Appl Physiol 107: 1591–1599, 2009 [PMC free article] [PubMed] [Google Scholar]
- Lalley PM. Opioidergic and dopaminergic modulation of respiration. Respir Physiol Neurobiol 164: 160–167, 2008 [PMC free article] [PubMed] [Google Scholar]
- Lara JP, Parkes MJ, Silva-Carvhalo L, Izzo P, Dawid-Milner MS, Spyer KM. Cardiovascular and respiratory effects of stimulation of cell bodies of the parabrachial nuclei in the anaesthetized rat. J Physiol 477: 321–329, 1994 [PMC free article] [PubMed] [Google Scholar]
- Lonergan T, Goodchild AK, Christie MJ, Pilowsky PM. Mu opioid receptors in rat ventral medulla: effects of endomorphin-1 on phrenic nerve activity. Respir Physiol Neurobiol 138: 165–178, 2003 [PubMed] [Google Scholar]
- Mansour A, Fox CA, Burke S, Akil H, Watson SJ. Immunohistochemical localization of the cloned mu opioid receptor in the rat CNS. J Chem Neuroanat 8: 283–305, 1995 [PubMed] [Google Scholar]
- Manzke T, Guenther U, Ponimaskin EG, Haller M, Dutschmann M, Schwarzacher S, Richter DW. 5-HT4(a) receptors avert opioid-induced breathing depression without loss of analgesia. Science 301: 226–229, 2003 [PubMed] [Google Scholar]
- Martin-Schild S, Gerall AA, Kastin AJ, Zadina JE. Differential distribution of endomorphin 1- and endomorphin 2-like immunoreactivities in the CNS of the rodent. J Comp Neurol 405: 450–471, 1999 [PubMed] [Google Scholar]
- Michelsen LG, Hug CC., Jr The pharmacokinetics of remifentanil. J Clin Anesth 8: 679–682, 1996 [PubMed] [Google Scholar]
- Michelsen LG, Salmenpera M, Hug CC, Jr, Szlam F, VanderMeer D. Anesthetic potency of remifentanil in dogs. Anesthesiology 84: 865–872, 1996 [PubMed] [Google Scholar]
- Montandon G, Qin W, Liu H, Ren J, Greer JJ, Horner RL. PreBotzinger complex neurokinin-1 receptor-expressing neurons mediate opioid-induced respiratory depression. J Neurosci 31: 1292–1301, 2011 [PMC free article] [PubMed] [Google Scholar]
- Mustapic S, Radocaj T, Sanchez A, Dogas Z, Stucke AG, Hopp FA, Stuth EA, Zuperku EJ. Clinically relevant infusion rates of mu-opioid agonist remifentanil cause bradypnea in decerebrate dogs but not via direct effects in the pre-Botzinger complex region. J Neurophysiol 103: 409–418, 2010 [PMC free article] [PubMed] [Google Scholar]
- Nicholson C. Diffusion from an injected volume of a substance in the brain tissue with arbitrary volume fraction and tortuosity. Brain Res 333: 325–329, 1985 [PubMed] [Google Scholar]
- Pattinson KT, Governo RJ, MacIntosh BJ, Russell EC, Corfield DR, Tracey I, Wise RG. Opioids depress cortical centers responsible for the volitional control of respiration. J Neurosci 29: 8177–8186, 2009 [PMC free article] [PubMed] [Google Scholar]
- Poisnel G, Quentin T, Barre L, Coquerel A, Debruyne D. Competitive displacement binding assay on rat brain sections and using a beta-imager: application to mu-opioid ligands. J Neurosci Methods 154: 60–67, 2006 [PubMed] [Google Scholar]
- Ren J, Ding X, Funk G, Greer J. Ampakine CX717 protects against fentanyl-induced respiratory depression and lethal apnea in rats. Anesthesiology 110: 1364–1370, 2009 [PubMed] [Google Scholar]
- Sales N, Riche D, Roques BP, Denavit-Saubie M. Localization of mu- and delta-opioid receptors in cat respiratory areas: an autoradiographic study. Brain Res 344: 382–386, 1985 [PubMed] [Google Scholar]
- Saper CB. The central autonomic nervous system: conscious visceral perception and autonomic pattern generation. Annu Rev Neurosci 25: 433–469, 2002 [PubMed] [Google Scholar]
- Song G, Poon CS. Lateral parabrachial nucleus mediates shortening of expiration during hypoxia. Respir Physiol Neurobiol 165: 1–8, 2009 [PMC free article] [PubMed] [Google Scholar]
- Stucke AG, Zuperku EJ, Sanchez A, Tonkovic-Capin M, Tonkovic-Capin V, Mustapic S, Stuth EA. Opioid receptors on bulbospinal respiratory neurons are not activated during neuronal depression by clinically relevant opioid concentrations. J Neurophysiol 100: 2878–2888, 2008 [PMC free article] [PubMed] [Google Scholar]
- Tonkovic-Capin M, Krolo M, Stuth EAE, Hopp FA, Zuperku EJ. Improved method of canine decerebration. J Appl Physiol 85: 747–750, 1998 [PubMed] [Google Scholar]
- Yassen A, Olofsen E, Dahan A, Danhof M. Pharmacokinetic-pharmacodynamic modeling of the antinociceptive effect of buprenorphine and fentanyl in rats: role of receptor equilibration kinetics. J Pharmacol Exp Ther 313: 1136–1149, 2005 [PubMed] [Google Scholar]
- Zadina JE, Hackler L, Ge LJ, Kastin AJ. A potent and selective endogenous agonist for the mu-opiate receptor. Nature 386: 499–502, 1997 [PubMed] [Google Scholar]
- Zhang Z, Xu F, Zhang C, Liang X. Activation of opioid mu receptors in caudal medullary raphe region inhibits the ventilatory response to hypercapnia in anesthetized rats. Anesthesiology 107: 288–297, 2007 [PubMed] [Google Scholar]